Nathan Schine Twists Photons and Cools Atoms in a Unique Quantum Dance
Deepening our understanding of the quantum world and developing new tools to peer into it is a very active area of physics research today. In this crowded field full of diverse theoretical ideas and physical tools, Assistant Professor and JQI Fellow Nathan Schine has managed to carve out a distinctive space for himself and his lab.
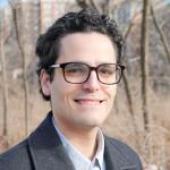
Schine’s research program manipulates the interactions between atoms and photons—the particles that make up light—in novel, well-controlled ways in order to simulate other, harder-to-probe quantum phenomena. To coax the photons into new simulation patterns, Schine is using unique arrangements of mirrors to bounce photons around. He is also strategically placing atoms in the photons’ way with the help of precisely controlled laser beams. To boot, the atoms he is using (ytterbium) have a relatively complex structure, giving Schine extra avenues to explore. He has been able to create this unique niche by combining the experimental expertise he gained from graduate school and postdoctoral research with his theoretical big-picture savvy.
Schine has been slowly homing in on his academic sweet spot for much of his life. Growing up, his interests were broad—they included science and math, but also history and other areas of the humanities. “It wasn't like I knew from an early age that I was going to go be a physicist,” Schine says.
Science wasn’t outside the realm of Schine’s imagination, however. His father was a chemistry teacher, his mother had a degree in math, and his grandfather was a physics professor at Vanderbilt University.
Keeping his options open, Schine attended Williams College. Ranked first among U.S. liberal arts colleges by U.S. News and World Report, Williams boasts an unusually strong science and math program. Schine was interested in math, but eventually found it to be too abstract for his taste. “When math got into proving the existence of a solution to a problem and not actually solving the problem, I sort of lost the thread a bit,” he recalls. Instead, he found that the part of math he enjoyed most could be gotten through physics, so he dove deeper into the subject.
An undergraduate research project sealed the deal for Schine as a physicist and experimentalist. He started working in the lab of his soon-to-be quantum mechanics professor, Barclay Jermain Professor of Natural Philosophy at Williams Protik Majumder, midway through his sophomore year.
Under Majumder’s supervision, Schine started to get a taste for experimental physics. He was performing spectroscopic measurements on indium atoms as a sophomore and continued working with Majumder until he graduated. Indium, with its three loosely bound, outermost electrons, is hard to model theoretically, and Majumder’s lab collaborated with theorists to benchmark their calculations and zero in on precise values.
Schine relished the chance to make a real contribution to the project. He also found joy in tinkering in the lab, finding his calling as an experimentalist. “I liked the day-to-day aspects of it, the actual process of building a laser or something,” Schine says. “A lot of it is very tactile and building up this sort of Rube Goldberg device that happens to be useful for physics—that, I think, is a lot of fun.”
Majumder had a slightly different take on what set Schine apart in his lab. “He was really unusual, even as a 20-year-old, in being able to balance comfortably the very hands-on build stuff with the bigger intellectual picture, which is obviously something that's been characteristic of his career since then,” Majumder says. Schine’s research with Majumder culminated in a senior thesis and a peer-reviewed publication.
Schine was inspired by his undergraduate research experience and decided to pursue graduate school. His chops setting up lasers and other experimental equipment meant he could hit the ground running and start contributing right away to the brand-new lab of Jonathan Simon at the University of Chicago.
The lab Simon was envisioning involved filling an optical cavity—a set of mirrors trapping light and bouncing it back and forth between them—with ultracold rubidium atoms. The idea was to use the photons themselves as a quantum playground, used to re-create and study quantum phenomena that happen in other, less accessible settings.
A lot of the interesting quantum phenomena that appear in real materials are hard to peer into at the quantum level but are nevertheless important for our daily lives because of their ubiquitous applications in technology. In Simon’s lab, precisely controlled photons can play a similar role to electrons inside of a material. Studying how these photons behave in a cavity and measuring them directly can then give clues about what happens inside the chunks of material.
There is one obvious limitation for photons playing the part of electrons: They don’t have an electric charge. And charged electrons—specifically in magnetic fields—are responsible for a range of interesting material effects that might need simulating.
Back in the early 1980s, physicists discovered one such effect. A thin layer of semiconductor placed inside a strong magnetic field was found to conduct electricity in very precise chunks. As the magnetic field is increased, the conductivity doesn’t change for a while—it stays at one plateau—and then hops abruptly to another plateau. This is known as the integer quantum Hall effect (IQHE) because the plateaus appeared at very regularly spaced integer values.
Even more strangely, for very cleanly engineered semiconductors, experimentalists found sub-plateaus within the plateaus, appearing at precise fractions of the previous integer values. They termed this, predictably, the fractional quantum Hall effect (FQHE). The origins of these fractional plateaus are largely still a mystery, although physicists are pretty certain that it has something to do with interactions between electrons giving rise to unexpected collective behaviors. If there was a way to simulate the full quantum theory of the FQHE, it might reveal new insights into what’s going on.
Simon and Schine, along with their labmates, hatched a plan. They conceived of a new way to make photons behave as though they have charge and live in a magnetic field that could, in principle, allow the photons to interact with each other and simulate the FQHE. Their plan involved a wonky cavity: four mirrors aligned to bounce light around in a twisted bow-tie configuration over and over again, with one of the mirrors slightly askew, as in the diagram shown below.
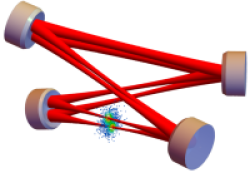
Photons and atoms in Schine’s tilted bow-tie cavity. (Credit: Nathan Schine/JQI)
Schine and his labmates focused on what happened along a plane at the center of this cavity. There, the photons were analogous to electrons traveling inside a thin material like in either of the Hall effects. The twisted mirror configuration causes the photons to twist around, much like electrons precess around inside a magnetic field.
With careful cavity design, they were able to make the analogy come to life and make their photons replicate the IQHE in its full glory. They published this result in the journal Nature.
To go beyond integer quantum Hall physics, the particles need to interact with one another—not just pass through each other, like photons are wont to do. That’s where atoms entered the picture. Previously, scientists had worked out a technique that allows atoms to serve as an intermediary through which photons can talk to each other.
In parallel with the twisted cavity work, Simon’s lab had been working on this atom-assisted approach to making photons interact with each other. This involved cooling a gas of rubidium atoms to extremely low temperatures, just a touch above absolute zero. Then, the light was tuned to a particular color that would allow one of the rubidium atoms to absorb a single photon. This atom then prevented any nearby atoms from absorbing a photon, ensuring no other photon got too close. This created an effective interaction between photons, where they were averse to being too close to one another.
The next step was to combine the two techniques: put the rubidium inside the skewed bowtie cavity. The cavity makes photons act like electrons in a magnetic field, and the atoms create a medium through which the photons can interact. The combination created the right conditions for FQHE physics. Although short-lived, the photons in Schine’s experiment appeared to indeed exhibit the hallmarks of fractional quantum Hall physics. Schine and his labmates published this result in the journal Nature.
This was the first time the fractional quantum Hall effect had been simulated in any medium. For his graduate work, Schine was named a finalist for a thesis prize from the American Physical Society’s Division of Atomic Molecular and Optical Physics, the most prestigious thesis award in this field.
Schine was still circling around his ultimate niche, though, and he sought to broaden his experimental skillset during his postdoctoral studies. He joined the group of Adam Kaufman at JILA at the University of Colorado Boulder (sometimes snarkily called JQI West). Kaufman’s lab manipulates atoms with light, using a tool called optical tweezers—laser beams focused down to a very narrow spot, intense enough to hold an atom in place.
Schine, Kaufman, and collaborators used these optical tweezers to put a new spin on atomic clocks, which are the most precise timekeepers we have. They work by counting the intrinsic ticking of individual atoms. Precise as they are, scientists are actively working on making them even more so, both for better technology like navigation and geolocation and for scientific inquiries, like the basic nature of fundamental constants and gravity.
The team endeavored to use a fundamentally quantum property—entanglement—to make pairs of atoms tick in tandem, thereby making the clock more precise. They cooled a gas of strontium atoms just above absolute zero and used optical tweezers to create a large array of atom pairs. These pairs were then made to interact using the same trick Schine had used during his graduate work: one atom absorbing a photon prevented another atom nearby from doing so as well, thus making their behavior depend on one another. Generating entangled atoms like this is a promising way to improve clock performance. They published this work in the journal Nature Physics.
Now, Schine is starting to build up his own lab here at the University of Maryland. When deciding exactly what kind of experiment to embark upon, Schine was guided by his graduate school advisor Simon’s philosophy. “There are different strategies for setting up experiments,” Schine says. “But I think Jon’s was very much to build something that no one else has done before experimentally—to put ourselves in an area where there's a lot of low-hanging fruit.” Schine explained that this will involve combining his optical cavity expertise with an array of tweezer-trapped atoms, now using ytterbium. For instance, he predicts this will allow dramatic improvements in performing quantum measurements, which is an essential part of quantum computing or quantum simulation experiments.
As Schine is assembling his lab and unique research program, he encourages interested students and postdocs to reach out to him. And, according to Schine’s undergraduate adviser, Schine’s teaching and mentoring abilities promise to be excellent. “One of the things we really work hard at in a place like Williams,” Majumder says, “is to make sure our students are not just the ones who can get into the lab, hide in a corner and just do amazing work. And that really comes through with Nathan. He's just such a good explainer of what he's doing. And he's so enthusiastic—it's very infectious.”
Story by Dina Genkina