The Chirps Heard Round the World
The collision of two black holes—a tremendously powerful event detected for the first time ever by the Laser Interferometer Gravitational-Wave Observatory, or LIGO on September 14, 2015—is seen in this still from a computer simulation. LIGO detected gravitational waves, or ripples in space and time generated as the black holes spiraled in toward each other, collided, and merged. Illustration: SXS.
Two black holes, locked together in close orbit for eons, abruptly ended their dance in spectacular fashion about 1.3 billion years ago. In two-tenths of a second, the pair of objects—about 29 and 36 times the mass of our sun—drew closer together, accelerated and merged to form a single black hole. The cataclysm instantly obliterated three suns’ worth of mass and transformed it into gravitational energy, which radiated outward in waves traveling at the speed of light, warping the fabric of space and time along the way.
These gravitational waves reached Earth on September 14, 2015. More to the point, they reached the twin detectors of the Laser Interferometer Gravitational-Wave Observatory (LIGO): one in Hanford, Washington, and another in Livingston, Louisiana. The waves altered the distances between LIGO’s mirrors by about one-thousandth of the width of a proton—just enough for the freshly upgraded detectors to take notice. A second event, the merger of two smaller black holes, set off the LIGO detectors again on December 26, 2015.
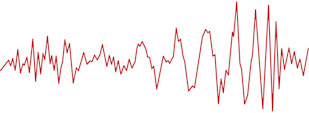
The “chirps” registered by LIGO simultaneously proved Albert Einstein to be both right and wrong. While his theory of general relativity predicted that gravitational waves exist, Einstein himself did not believe humans could ever build a detector sensitive enough to observe them.
The LIGO team’s discoveries marked the pinnacle of a scientific quest more than a half-century in the making. By twice detecting gravitational waves, LIGO earned its status as a true observatory and ushered in a new age of gravitational wave astronomy. Now, astrophysicists no longer need to rely solely on electromagnetism—radio waves, visible light, X-rays and gamma rays—to observe some of the most explosive events in the cosmos.
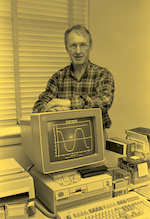
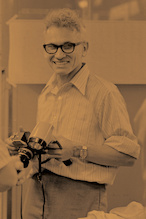
The historic detections resulted from the hard work and insights of more than a thousand scientists, engineers and technicians from around the world, including many affiliated with the University of Maryland. In fact, the effort to observe gravitational waves began more than 50 years ago in the College Park laboratory of the late Joseph Weber.
Widely regarded as the first to accept Einstein’s challenge, Weber launched the first concerted effort to detect gravitational waves. His tenacity and passion sowed the seeds for a vibrant gravitational research group at Maryland that carries on to this day.
Around the time Weber began his experiments, he worked with Physics Department Chair John Toll to recruit Charles Misner—already a big name in gravitational theory—to Maryland from Princeton. At UMD, Misner mentored an entire generation of talented gravitational theorists, including many who went on to make direct or indirect contributions to the LIGO effort. The successful combination of experimentation and theory at Maryland created a rich academic environment matched by few other institutions at the time.
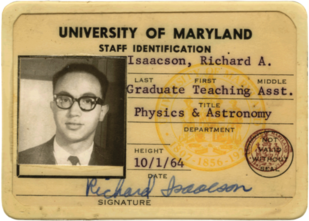
“Maryland was one of the only places where gravitational work was being done both theoretically and experimentally. It was a real center of thought,” says Richard Isaacson, Ph.D. ’67, physics, a student of Misner’s who went on to steer the LIGO funding effort as a program officer at the National Science Foundation (NSF). “At a time when general relativity existed solely in the realm of math and theory, Weber was one of the first to think we could see gravitational waves experimentally. And Misner was a fabulous mentor who got the absolute best out of each one of his students.”
UMD’s influence in gravitational research continues today, with alumni and current faculty members engaged in the LIGO project and related work. As the quest for gravitational wave observation gives way to the age of gravitational wave astronomy, UMD scientists and alumni continue to lead the way with new technical innovations and theoretical insights.
Stages of a Black Hole Merger: The waveforms from both LIGO sites recorded three characteristic phases of a black hole merger on September 14, 2015. During the inspiral phase, both black holes accelerated to nearly the speed of light as their orbits drew closer together. The final merger sent a powerful surge of gravitational waves as three suns’ worth of mass was instantly converted to gravitational energy. A rapid decline in the signal intensity marked the ringdown phase, when the newly created single black hole regained a stable equilibrium. Illustration: LIGO, NSF, Aurore Simonnet (Sonoma State U.)
#EinsteinWasRight
The LIGO Scientific Collaboration and the NSF announced the first detection of gravitational waves at a press conference on February 11, 2016. Almost immediately, scientists and enthusiasts all over the world took to Twitter, celebrating with the hashtag #EinsteinWasRight. Their excitement was well warranted; for the first time, physicists had direct experimental evidence for the last remaining major theoretical prediction of Einstein’s theory of general relativity.
Einstein published his theory, known to most physicists simply as “GR,” in 1915. More than a century later, GR remains the best description of gravity’s behavior available to science. It has repeatedly stood up to theoretical challenges, and most of its tenets—including the idea that the gravity of massive objects can observably warp space and time—have been verified by experimentation. But the fact that gravity itself can travel through space and time in the form of waves had yet to be directly observed.
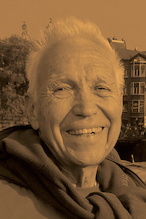
“With Einstein, there were certain things he didn’t have any doubt about. To him, it was almost irrelevant whether gravitational waves could be confirmed experimentally, because he knew the theory was right,” says Dieter Brill, professor emeritus of physics at UMD who overlapped with Weber and Misner and also mentored a number of talented gravitational theorists.
Early in his career, Brill helped build a theoretical case that gravitational waves did carry energy—and thus could be observed with a sufficiently sensitive instrument.
“I didn’t share Einstein’s dismissive feelings about the necessity of detecting gravitational waves. It was really a wonderful thing to see them confirmed,” Brill adds.
The sense of excitement and accomplishment rippled throughout the scientific community, including UMD’s students, alumni, and current and emeriti faculty.
“My preferred name for this first big event is the ‘Hallelujah Chirp,’” says Misner, professor emeritus of physics. “Thousands of people had been working on this for decades and, until now, nothing had come from it. It was a great relief when finally something appeared. LIGO did what it was designed to do. I was madly excited when I heard the news.”
For Alessandra Buonanno, a UMD College Park Professor of Physics and LIGO principal investigator, the results were monumental.
“What we’re observing with LIGO is so spectacular. It stands next to many of the other great things human beings can do—like any great masterpiece in music or art,” says Buonanno, who also has an appointment as director at the Max Planck Institute for Gravitational Physics in Potsdam, Germany. “The fact that we can detect an event that lasted a fraction of a second, far across the universe, is amazing. It’s almost magical.”
The House that Weber Built
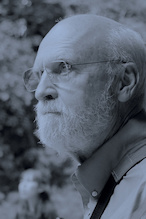
At the February 2016 press conference, LIGO Co-founder Kip Thorne of Caltech name-checked UMD’s Joseph Weber as a pioneer of the search for gravitational waves. It was an expected and appropriate nod, given Weber’s undeniable contributions to the field. However, Weber’s achievements and leadership are often overshadowed by another aspect of his legacy: he claimed to have detected gravitational waves in 1969, but despite a flood of efforts, no other researchers were able to replicate his results.
“I think Weber did truly believe that he had found gravitational waves,” says Misner. “But either way, he absolutely inspired a lot of other people when he claimed discovery. Everyone will agree that he did a great service because he gave us hope.”
Weber not only spurred experimentalists to follow suit in his search for direct evidence, but also drove many important theoretical advances that aimed to predict the behavior of gravitational waves.
“Weber certainly invented the field. I can’t think of anyone else who had the idea that you could actually detect gravitational waves,” says Richard Matzner, Ph.D. ’67, physics, a professor of physics at the University of Texas at Austin. Matzner, one of Misner’s students, made some of the earliest calculations of what a simplified head-on collision between two black holes would look like, including the weak gravitational waves such a merger could emit.

“Weber thought it was a tractable problem. Everyone stands on the shoulders of giants, and Weber is one of them,” says Matzner.
Initially a professor of electrical engineering at UMD, Weber moved to physics in 1961. He began investing the majority of his time building resonant bar detectors—giant masses of aluminum weighing more than a ton that he expected would “ring” like a bell when struck with gravitational waves. Weber placed an array of sensors around the outer surface of the bars to catch any small change in the shape of the bars.
Weber built one such bar detector in a small, climate-controlled building on the UMD golf course, and he tended the equipment almost daily with the help of his dedicated engineering technician, Darrell Gretz. Eventually, Weber recognized the need for two identical detectors, separated by several hundred miles. Such “coincidence experiments” would help identify real gravitational wave signals amid considerable background noise from passing trucks, powerful storms and other events capable of rattling the detector. So, Weber installed a second detector at Argonne National Laboratory near Chicago.
“Weber supplied the brains and the money, and I was in charge of building, maintaining and operating the equipment,” says Gretz, who worked with Weber for nearly 25 years. “At the time I never gave it a second thought, but if he was in the lab, I was always there looking over his shoulder.”
John Giganti, B.S. ’66, M.S. ’70, electrical engineering, helped develop an efficient communications link for sharing data between the College Park and Argonne locations. Long before the advent of the internet, Weber’s team shared data across hundreds of miles.
“Our system had a relatively high data rate,” explains Giganti, who recently founded his second engineering and design firm. “Telephone lines today are designed for data, but back in the ’60s they were designed for talking to grandma. So we digitized the data and sent it over separate communication lines.”
![]()
![]()
![]()
![]() |
Weber also attracted other talented experimentalists to work with him at UMD, including Jean-Paul Richard, who came to Maryland in 1965 and is now an emeritus professor of physics. For his first project, Richard designed temperature control shielding for the Lunar Surface Gravimeter that flew on the Apollo 17 mission in 1972. The instrument’s intended purpose was to look for gravitational waves, but a technical issue meant that the unit was able to function best as a sensitive seismograph.
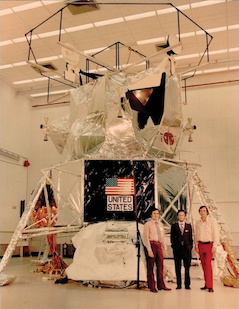
Until his retirement in 1998, Richard worked on various technological improvements to resonant bar detectors like those designed by Weber, including more efficient resonators and transducers that could expand the detectors’ sensitivity. For Richard, LIGO’s discovery provided some important validation.
“I worked from 1965 to 1998 without finding anything. I retired with misgivings as to whether my work would ultimately be useful,” Richard says. “When I heard the LIGO news, I was shocked and stunned for a couple of days. It gave new value to my work and justified my efforts. That’s a very big thing."
In 1978, at Richard’s urging, Weber recruited a talented young postdoctoral researcher named Ho Jung Paik, who had recently finished his Ph.D. at Stanford University. Paik, now a professor emeritus of physics who continues to run an active research program at UMD, designed the first cooled, superconducting transducers to help boost the signal of resonant bar detectors like those designed by Weber. In theory, such upgrades were capable of improving the detectors’ sensitivity nearly a thousandfold.
“Unfortunately, bar detectors are difficult to scale up, which meant that they would always be limited in size. Then, the laser interferometer was proposed in the 1970s,” Paik explains. “Laser light overcame the size problem because it could extend the detector’s baseline from a few meters to several kilometers. NSF began funding interferometer work in a major way in the 1980s. We all could see the writing on the wall.”

Weber worked on some of the earliest laser interferometer designs, with help from the late Robert Forward, B.S. ’54, Ph.D. ’65, physics—who eventually became a well-known science fiction writer. Forward was among the first to clearly articulate the potential of laser interferometers to detect a wider range of frequencies compared with resonant bar detectors. Forward developed his own interferometer designs while working at Hughes Aircraft Company in California in the late ’60s.
Despite the promise offered by laser technology, Weber remained dedicated to his resonant bar detectors until his death in 2000.
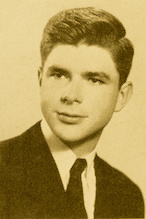
“Joe knew that you had to have two detectors and introduce a time delay between the data streams to measure the rate of accidental coincidences between the detectors. LIGO does both of these things,” says Virginia Trimble, Weber’s widow and a professor of physics and astronomy at the University of California, Irvine. She was a visiting professor of astronomy at UMD from 1973 to 2003. “Joe was certain his bars were detecting something and almost certain that it was gravitational radiation. If asked, he would probably have said that he always knew the waves existed and that the discovery would have occurred earlier if both technologies, bars and interferometers, had been funded and developed in parallel.”
Lighting the Path
What began as sketches on paper quickly turned into proof-of-concept experiments, with a trio of scientists lighting the path that would eventually result in LIGO: Kip Thorne and Ronald Drever of Caltech and Rainer Weiss of MIT. The project gained steam throughout the 1980s, suffering some setbacks but ultimately receiving its first significant funding award from NSF in 1992.
A view of the LIGO detector in Hanford, Washington. A single laser beam is split in two and each half travels along one of two perpendicular, 2.5-mile long “arms” that form the interferometer’s characteristic “L” shape. A mirror at the end of each path reflects each beam back toward the original source, where the beams recombine and enter a light detector. When a gravitational wave passes, the lengths of the arms change by a minuscule amount, causing the recombined beam to flicker. LIGO’s light detectors record this oscillation, enabling the LIGO team to reconstruct the shape and strength of the incoming gravitational wave. Credit: NSF.
The basic concept behind a laser interferometer is this: A single laser beam is split in two, and each half of the beam travels along one of two pathways that form the arms of the interferometer’s characteristic “L” shape. A mirror at the end of each path reflects each beam back toward the original source, where the beams recombine and enter a light detector. When there is no gravitational wave present, the split beams each travel a fixed distance, and the light detector measures a constant intensity.
But when a gravitational wave sweeps by, it changes the lengths of the arms by a minuscule amount, and the recombined beam flickers ever so slightly. LIGO’s light detectors record this oscillation, enabling software to reconstruct the shape and strength of the incoming gravitational wave itself. Millisecond differences in the wave’s arrival time at the Hanford and Livingston detectors can help determine roughly where the wave came from.
Both gravitational wave events observed by LIGO lasted a second or less. But in that time, the twin LIGO detectors collected enough data to paint an astonishingly clear picture of what each stage of a black hole merger looks like.
“Laser technology provided a broadband view. Instead of being able to hear just one tone, one could hear the whole symphony. I had been selling the NSF on the fact that this was actually doable and that there would be great payoffs,” says Isaacson, whose efforts at NSF were crucial to LIGO’s funding and eventual construction.
“We not only had to push the technology to build instrumentation, we also knew we had to have supercomputers and good models to make the data useful,” Isaacson says. “There also wasn’t a large community with people ready to step into leadership roles. I don’t think people understand how crazy this was. We had just the right window of opportunity.”
Paik and his physics colleagues, recognizing a need for UMD to be involved in the LIGO effort, advertised a new LIGO-specific faculty position and ultimately hired Peter Shawhan in 2006. Now a LIGO principal investigator and associate professor of physics at UMD, Shawhan had been working on the project for seven years as a postdoctoral researcher at Caltech.
“That was still a fairly early stage. The project was funded and the observatories were built, but the instruments were not yet installed,” Shawhan says. “We all had faith that we would get there someday. My role was to help figure out how we would monitor the detectors and analyze the data.”
One crucial innovation the LIGO team devised, with Shawhan’s help, was an elaborate “fire drill” system. Unbeknownst to other team members, a simulated signal would be randomly introduced into both detectors to test the team’s response and—crucially—data analysis protocols. The entire LIGO group learned of these “blind injections” only after completing a significant number of analysis steps. Although Shawhan helped to design the blind injection system, he did not know when a test was being performed.
“In 2010, we had a blind injection test that was so convincing, it looked very much like an event candidate,” Shawhan says. “We put a lot of effort into treating it as a real signal and got as far as preparing a paper for submission. We didn’t know ourselves whether the signal was real or another test. We didn’t want to risk letting the information leak out. This uncertainty gave us a built-in safety measure.”
Once the LIGO team recognizes a candidate signal, the hard work begins. Even the strongest gravitational wave signal is still extraordinarily weak compared with those commonly received by conventional telescopes and other instruments. Buonanno and her collaborators spent nearly a decade developing models to quickly and efficiently pull promising signals out from behind the wall of noise that obfuscates them.
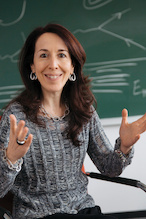
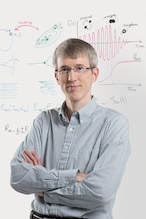
“Say you are in a room where there is a lot of ambient noise and people talking, but you want to select one of the voices,” Buonanno explains. “This is what happens in the detector. The signal is buried in the detector noise, so to pull it out we need some filters. We call them templates or waveform models.”
The process resembles the way a forensic scientist would compare a fingerprint found at a crime scene against a bank of known fingerprints.
“Each black hole in a binary contributes its own ‘fingerprint’ to the observed waveform,” says Buonanno. “So, by matching the observations to one of our waveform model templates, we can infer the properties of each black hole, including mass and spin.”
The problem, according to Buonanno, is that for any one given event, the LIGO team needs to check hundreds of thousands of potential templates to match the observed signal. To do this, the team must create a large bank of these waveform templates in advance, using equations based on known theory. The method developed by Buonanno and her colleagues enables the LIGO team to do exactly this, by combining the strengths of two different—but complementary—mathematical approaches.
“Alessandra has played an incredibly important role in the waveform modeling and data analysis,” says Misner. “She found a way to simplify the calculations by extrapolation. This is an essential step—almost as important as the hardware.”
Once an event is matched to a waveform template, physicists then have powerful clues to describe the event that created the gravitational waves. For the first two events detected by LIGO, the team discerned the masses of the black holes—both before and after the merger—and each event’s approximate distance from Earth.
The second event, known as GW151226 based on the date of its discovery, resulted from the merger of two black holes that were eight and 14 times the mass of the sun. Because of the smaller masses relative to the first event, the second merger converted only a single sun’s worth of mass into gravitational energy. But the event lasted about one full second—roughly five times longer than the first event—allowing the LIGO team to extract much more information from the data.
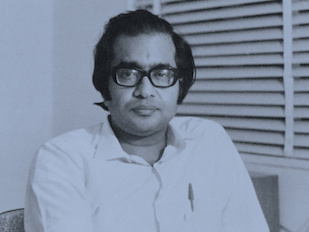
“GW151226 perfectly matches our theoretical predictions for how two black holes move around each other for several tens of orbits and ultimately merge,” says Buonanno. “Remarkably, we could also infer that at least one of the two black holes in the binary was spinning.”
The first event, GW150914, offered a look at what is known as the “ringdown” phase—the moment that immediately follows a black hole merger and the initial spike of gravitational energy, when the two objects quickly settle into a stable form as a new, larger black hole. C.V. Vishveshwara, Ph.D. ’68, physics, a theoretical physicist and director emeritus of the Jawaharlal Nehru Planetarium in Bangalore, India, who is best known for proving that non-rotating black holes are stable, first predicted the ringdown phase in the early 1970s.
“Gravitational waves and black holes both belonged to the realm of mythology,” Vishveshwara told the journal Nature in March 2016. “At that time, I had not imagined that [the ringdown phase] would ever be verified.”
A New Age of Gravitational Astronomy
It’s hard to overstate the significance of observing two gravitational wave events within months of each other.
“By December, we were sure that the first event was genuine,” Shawhan says. “But it was very satisfying to know, even then, that we already had a second event on our hands.”
Many have pointed out that the second observation gave true meaning to the “O” in LIGO, making it an observatory and not just a one-shot tool for a single discovery. There will almost certainly be more events detected in the near future, and LIGO’s initial successes will be remembered as the first of a new age of gravitational astronomy.
During LIGO’s next data-taking run, the team expects further improvements in detector sensitivity will allow LIGO to reach as much as 1.5 to two times more of the volume of the universe compared with the first run.
The Virgo detector, a third interferometer located near Pisa, Italy, with a design similar to the twin LIGO detectors, is expected to come online during the next observation run. Virgo will improve the ability to locate the source of each new event by comparing the arrival times of incoming gravitational waves at each detector.
But Virgo won’t be alone. The NSF recently signed a memorandum of understanding with the government of India to build a third LIGO detector, and a Japanese collaboration began constructing the Kamioka Gravitational Wave Detector (KAGRA) in central Japan. With three new detectors joining the hunt, physicists expect that they will soon be able to “hear” gravitational signals from a greater number and variety of events.
“The first detection gave us all an intimate connection to a specific event that took place 1.3 billion years ago, incredibly far away. This spectacular warping of space and time happened and essentially waved hello to us,” says Ted Jacobson, a physics professor and gravitational theorist at UMD who has not worked directly on the LIGO project. “These discoveries also herald a new era in science. It’s like someone who has never heard before suddenly can hear.”
Written by Matthew Wright
This article was published in the Fall 2016 issue of Odyssey magazine. To read other stories from that issue, please visit go.umd.edu/odyssey.